On-demand single-photon source
Atoms at room-temperature generate and store single photons enabling mass-deployable quantum repeaters.
In the field of quantum optics the single-photon state is a cornerstone. It is the state that describes a light pulse which contains exactly one photon. As such it is one of the fundamental quantum states that students will know from introductory quantum optics classes.
Even though the first reports of successful single-photon generation appeared more than two decades ago, it is still an outstanding goal to find sources that deterministically, as opposed to probabilistically, produce pure single photons – and are technologically simple.
In our lab we address this challenge by investigating single-photon generation in a glass container (aka. cell) enclosing a gas of Cesium atoms, referred to as an atomic ensemble. When illuminating the atomic ensemble with laser pulses, the light-atom interaction causes a few photons from the pulse to be scattered off the atoms. This leaves an imprint on the atomic spin which can then act as a memory for single photons. Exploiting the atomic memory enables on-demand release of single photons.
Herald and retrieve
The scheme we employ is a herald-retrieve scheme which is carried out in two steps.
1) Write:
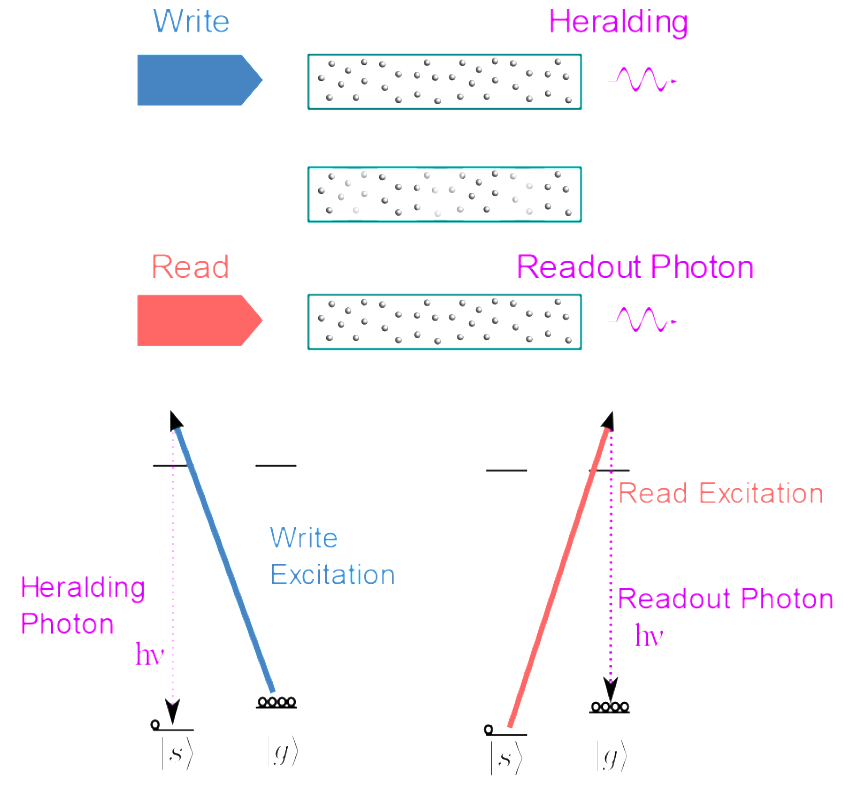
A first pulse of light interacts with the atoms and has a small probability of scattering photons. For every scattered photon one atomic spin excitation is created. This excitation is shared between all atoms illuminated by the pulse and involves a phase mark on the individual atoms dependent on position. Hence, this is often referred to as a spin wave. Since the spin wave excitations and the scattered photons are created in pairs, we can use the detection of a single scattered photon to herald the creation of a single atomic excitation. Or to put it in slightly different terms: The detection of a single scattered photon collapses the atomic wave function into the single-excitation state. Because number-resolved photon detection is very sensitive to photon loss (e.g. lossy transmission to detectors), we cannot distinguish between one or multiple scattered photons. Hence, the probability to scatter photons has to be kept very low (a few percent) to minimize the creation of multiple pairs of photons and spin waves. This makes step 1 inherently probabilistic.
Because our cells sustain long-lived spin states, the spin wave excitation has a lifetime on the order of milliseconds. This is the memory time during which we can wait until we decide to perform step 2 – the retrieval.
2) Read:
A second pulse of light interacts with the atoms and (near-)deterministically converts spin wave excitations into photons at any time within the memory time. The conversion is one to one, i.e. if we successfully herald a single spin wave excitation in step 1, we retrieve a single photon. Deterministic retrieval is possible due to an effect known as collective enhancement which arises from the spin wave being shared between all atoms of the ensemble.
The deterministic retrieval mitigates the probabilistic nature of the creation. If you want to conduct an experiment which relies on one mode containing a single photon, heralded probabilistic generation works just fine. However, if you rely on interfering multiple modes of single photons, the introduction of single-photon memory enables photon synchronization which greatly enhance the probability of photons arriving simultaneously at the interference point.
Another direct application of single-photon memory is in quantum repeaters. Quantum repeaters extend the distance over which entanglement can be distributed in a network and are a crucial elements of the future Quantum Internet.
Atomic motion - a foe becomes a friend
While a room-temperature gas offers a technically simple platform, it comes with the price of atoms always being in motion. Since atomic phase information is position dependent, atomic motion will quickly dephase (wash out) the spin wave. This holds true for all spin wave modes except one: the “symmetric” spin wave mode where the phase is constant and all atoms hold equal amounts of the excitation. Ironically, the interaction with the flat mode can be enhanced over all other modes by exploiting the atomic motion. If the light pulse is long enough for each atom to move in and out of the beam several times, they will all have experienced identical interaction with the light independent of individual atomic trajectories. We call this process “motional averaging” because atomic motion averages out differences in atom-light interaction.
The fundamental challenge of the single-photon experiment is to conserve the motional averaging while detecting the heralding photon. This requires erasing “which-atom” information of the scattered photon and is achieved through spectral filtering. On top of this, we also investigate methods to minimize atomic noise in order to eventually achieve pure single photon generation.
Researchers
- Rebecca Schmieg, PhD student
Former members:
- Isaac Roca Caritg, Master student
- Karsten B. Dideriksen, PhD student
- Michael Zugenmaier, Postdoc
Publications
- Dideriksen, K. B., Schmieg, R., Zugenmaier, M., Polzik, E. S. (2021). Room-temperature single-photon source with near-millisecond built-in memory, Nature Communications, 12, 3699
- Zugenmaier, M., Dideriksen, K. B., Sørensen, A. S., Albrecht, B., Polzik, E. S. (2018). Long-lived non-classical correlations towards quantum communication at room temperature. Communications Physics, 1, 76.
- Borregaard, J., Zugenmaier, M., Petersen, J. M., Shen, H., Vasilakis, G., Jensen, K., Polzik, E. S., Sørensen, A. S. (2016). Scalable photonic network architecture based on motional averaging in room temperature gas. Nature Communications, 7, 11356.