Magnetic induction tomography- measuring magnetic fields with atomic vapor cells
Optically-pumped atomic magnetometers show record sensitivities in measuring magnetic fields. We are exploring the quantum limits of these devices. Furthermore, we are investigating the application of these magnetometers in the bio-medical field.
Atomic magnetometers
Optically-pumped atomic magnetometers are high-sensitivity detectors of magnetic fields that employ the electron spin of cesium (or other alkali) vapor atoms as an extremely responsive magnetic compass. Optical pumping is used to generate spin polarization, which precesses to the external magnetic field. The precession (Larmor) frequency is directly related to the strength of the magnetic field. The precessing spin is detected optically, e.g. by Faraday rotation of a near-resonant probe beam. The magnetometer sensitivity is fundamentally related to the number density of the vapor and the atomic spin coherence time. To achieve good coherence times, the cell walls can be coated with paraffin-like anti-relaxation coatings, which enable the atoms to experience many wall collisions with no change in the spin state.
Magnetic Induction Tomography – imaging low conductivity
Detection and imaging of an electrically conductive object at a distance can be achieved by inducing eddy currents in it and measuring the associated magnetic field. Typical applications of this method are e.g. to detect cracks in metal plates or tubes. If a crack is present in a conductive material, the eddy currents cannot flow through it. The magnetic field associated with the eddy current changes and thus the signal that is typically picked up with a coil reveals the presence of the crack.
A different application is imaging the conductivity of biological tissue, e.g. the human heart. Here, the aim is not to detect cracks, but to detect tissue that has anomalous conductivity, disturbing the electrical impulses and leading to heart beat arrythmia. The challenge here is to image the heart tissue, having very low conductivity (<~1S/m).
We have detected low-conductivity objects with an optical magnetometer based on room-temperature cesium atomic vapor and a noise-cancelling differential technique which increased the signal-to-noise ratio (SNR) by more than three orders of magnitude. We detected small containers with a few mL of salt-water with conductivity ranging from 4-24 S/m with a good SNR. This demonstrates that our optical magnetometer should be capable of detecting objects with conductivity < 1 S/m with a SNR > 1 and opens up new ways for using optical magnetometers to image low-conductivity biological tissue including the human heart. This will enable non-invasive diagnostics of heart diseases.
The AC current in coil 1 creates a primary magnetic field B1. This induces eddy currents in the saltwater which in turn creates the magnetic field Bec. We measure the magnetic field Bec using the cesium (Cs) atom vapor cell as a magnetometer while the saltwater is at different positions along the x-axis. The coil 2 can be used to increase the SNR with a differential technique.
As the saltwater container is moved along the x-axis, we plot alpha, the strength of the induced field Bec relative to the primary field. The signal decreases with lower conductivity.
Magnetocardiography – measuring the heartbeat at a distance
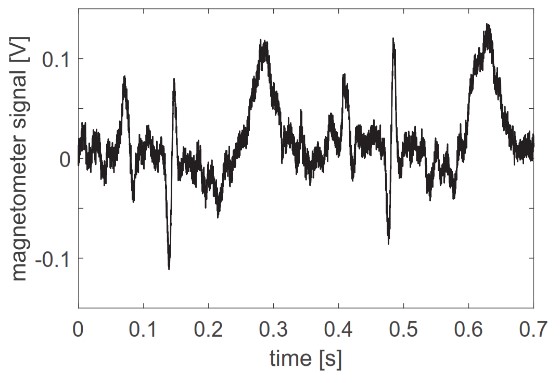
Example of a magnetometer signal (without averaging) in volts when a guinea-pig heart is placed close to the magnetometer
Magnetic field detection is a completely non-invasive method, which allows one to study the function of excitable human organs with a sensor placed outside the human body. For instance, magnetometers can be used to detect brain activity or to study the activity of the heart. We have developed a highly sensitive miniature optically pumped magnetometer based on atomic vapor kept in a paraffin-coated glass cell. The magnetometer is optimized for detection of biological signals and has high temporal and spatial resolution. It is operated at room- or human body temperature and can be placed in contact with or at a mm-distance from a biological object. With this magnetometer, we detected the heartbeat of an isolated guinea-pig heart, which is an animal widely used in biomedical studies. In our recordings of the magneto-cardiogram, we can in real-time observe the different stages (P-wave, QRS-complex and T-wave) of the heartbeat.
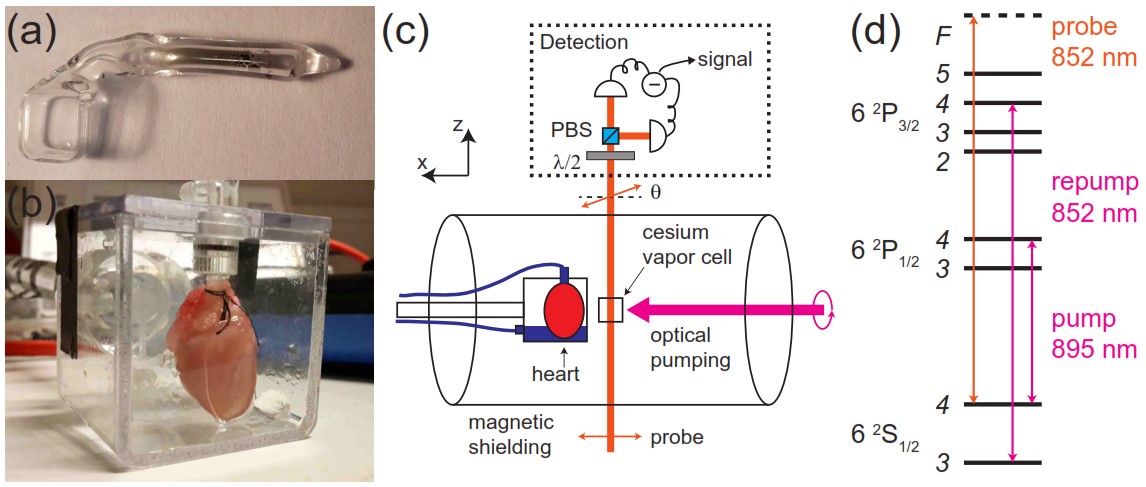
Experimental setup: (a) Picture of the cesium vapor cell. (b) Picture of an isolated guinea-pig heart inside a plastic chamber. (c) Schematics of the experimental setup. λ/2: half-wave plate, PBS: polarizing beamsplitter. The magnetic field from the heart affects the cesium atomic spins which in turn rotates the polarization of the probe light by an angle θ. This polarization rotation is detected with a balanced polarimeter (shown inside the dashed-line box). (d) Cesium atom level scheme and laser frequencies.
Quantum-enhanced MIT
Currently, we are investigating how we can exploit quantum mechanical effects such as spin-squeezing to enhance the sensitivity of our atomic magnetometer beyond the level attainable by classical means. Fundamentally, atomic magnetometry and hence MIT are fundamentally limited in their performance by the so-called standard quantum limits of measurement sensitivity - SQLs. For a continuously operating MIT measurement, the SQL is set by the measurement itself and its disturbance caused in the measurement system, in our case the collective spin. Our measurement suffers from three different noise contributions originating from the shot noise of light, the coupling to the atomic projection noise and the back-action noise of the light back onto itself. To overcome the SQL of a continuous measurement, we investigate quantum mechanical effects and try to exploit them to reduce the noise introduced to our system through our measurement. Our approach includes stroboscopic back-action evasion and conditional spin-squeezing. The former can be understood as probing our system in a smart way to avoid introducing detrimental back-action noise. The latter, conditional spin-squeezing, corresponds to exploiting a second measurement on a preceding one. This squeezes the uncertainty in the measurement observable.
Combining these resources, we reduce the measurement noise in our system below the SQL of a continuous MIT and verify this by observing a noise reduction when detecting the presence of a conductive sample.
Researchers
- Rebecca Schmieg, PhD student
- Veronika Kaminski, Master student
Former members:
- Michael Zugenmaier, Postdoc
- Alan Oesterle, Master student
- Wenqiang Zheng, Postdoc
- Hengyan Wang, Postdoc
Publications
- Detection of low-conductivity objects using eddy current measurements with an optical magnetometer, Kasper Jensen, Michael Zugenmaier, Jens Arnbak, Hans Stærkind, Mikhail V. Balabas, and Eugene S. Polzik, Phys. Rev. Research 1, 033087 – Published 8 November 2019
- Jensen, K., Skarsfeldt, M.A., Stærkind, H. et al. Magnetocardiography on an isolated animal heart with a room-temperature optically pumped magnetometer. Sci Rep 8, 16218 (2018). https://doi.org/10.1038/s41598-018-34535-z
- Jensen, K., Budvytyte, R., Thomas, R. et al. Non-invasive detection of animal nerve impulses with an atomic magnetometer operating near quantum limited sensitivity. Sci Rep 6, 29638 (2016). https://doi.org/10.1038/srep29638